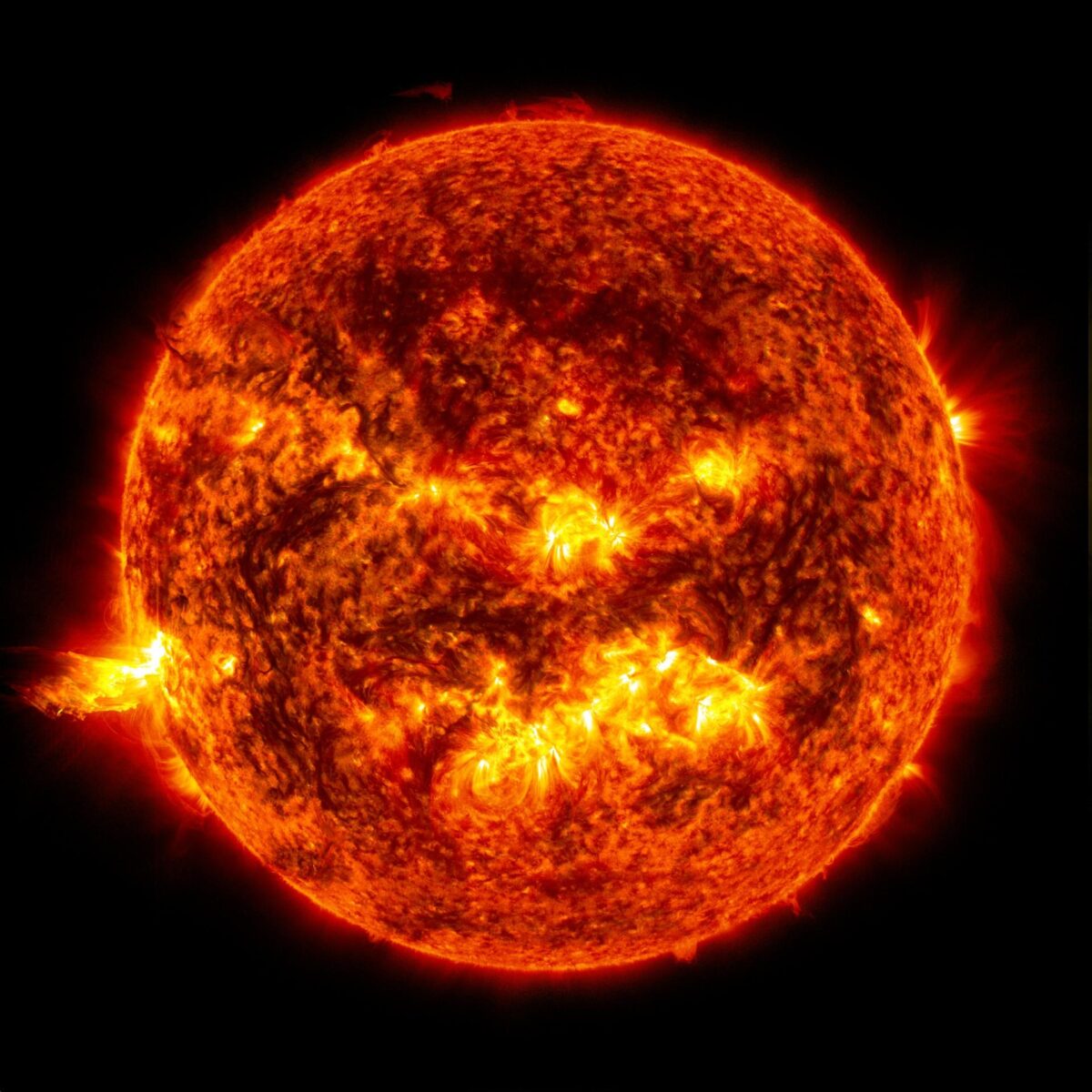
For thousands of years, humans have worshipped the Sun. Our ancestors built monuments and temples to it, and used it to mark the annual cycle of seasons. For ancient Egyptians, their most important god, Re, was the personification of the Sun itself.
Today, we are no less in thrall to the wonders and mysteries of our nearest star. We’ve made strides in understanding its major systems and answered many questions about how it produces energy. But the Sun is far from an open book, concealing countless conundrums: its hidden deep core, its roiling layers of gas, its ethereal outer atmosphere, its writhing magnetic field. Solar physicists still cannot fully explain phenomena like the eruption of solar storms or how the corona reaches temperatures of millions of degrees.
Understanding these mysteries is not just an academic matter. In truth, we are just as dependent on the Sun as the ancients were — and in some ways, our modern civilization is even more vulnerable to its heated wrath.
The core of the matter
For millennia, our studies of the Sun were limited to what could be gleaned from observations of its exterior. The earliest records of sunspots are found in China around 700 b.c.e. In the 17th century c.e., telescopic observations of sunspots revealed that the Sun’s poles rotate slower than its equator, a phenomenon called differential rotation. By the 19th century, observers had also deduced a cycle in the appearance of sunspots and noted that their appearance was correlated with geomagnetic storms on Earth and displays of the aurora.
By the early 20th century, researchers understood that the source of the Sun’s energy had to be something truly remarkable. Advances in geology told them that the lifespan of Earth — and therefore the Sun — was measured in billions of years. But all known chemical and gravitational sources of energy were too short-lived.
A revolution came in the 1930s, when nuclear fission and fusion were discovered with help from Einstein’s iconic E = mc2. Combining principles of nuclear physics with the observed abundance of hydrogen in the Sun, physicists Hans Bethe and Charles Critchfield proposed in 1938 that the Sun’s energy was the result of fusion — predominantly, a proton–proton chain reaction.
Hotter and more massive stars, the physics held, use either a second cycle called the carbon-nitrogen-oxygen (CNO) cycle, or a third, called the triple-alpha process.
According to nuclear theory, these processes produce neutrinos — ghostlike particles that can zip through light-years’ worth of matter without being absorbed. Solar neutrinos fly through Earth at nearly the speed of light about 8.5 minutes after they are created in the Sun’s core. And in fact, the only direct proof that these fusion cycles were occurring relied on the detection of these neutrinos.
A race began to build detectors capable of seeing these particles. Physicists Raymond Davis Jr. and John Bahcall began monitoring the Sun for neutrinos in the late 1960s. Solar neutrinos were detected, but at only a fraction of the numbers per day that were expected — a situation that became known as the solar neutrino problem.
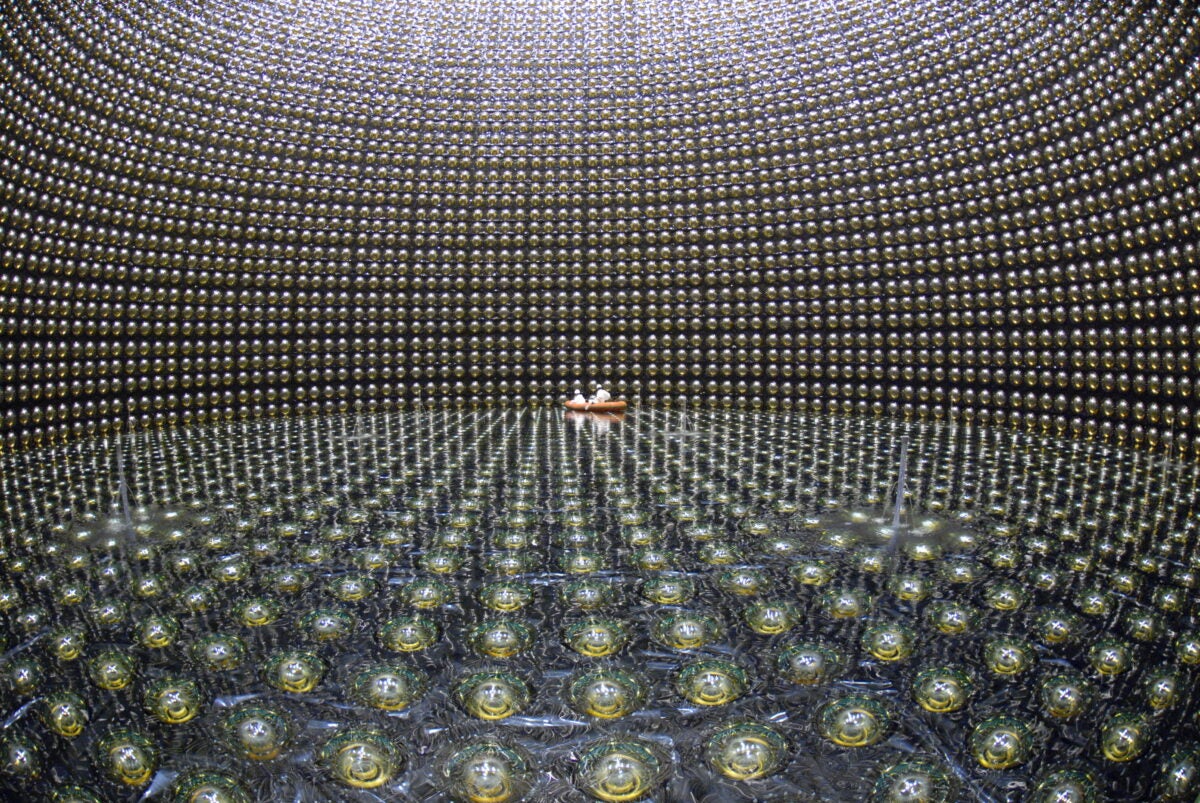
It turns out neutrinos come in three flavors: electron, muon, and tauon. A single neutrino can be any of these flavors as well as change between them, so a detector must be able to capture all three types to get an accurate tally. The “missing” neutrinos were found once more sensitive detectors like the Sudbury Neutrino Observatory in Ontario and the Super-Kamiokande Observatory in Japan came online in the 1990s, leading to the discovery of this interchange, called neutrino oscillation. And with a full picture of the Sun in neutrinos came confirmation that thermonuclear hydrogen fusion was going on in its core.
Recently other discoveries about the Sun’s core have come to light. In 2017, a team led by Eric Fossat at the Côte d’Azur Observatory in France used 16 years of data from the Solar and Heliospheric Observatory (SOHO) to detect waves traveling through the Sun’s interior. These gravity-mode (or g-mode) oscillations are caused by blobs of plasma rising quickly through the Sun, then sinking again once they reach a region with lower density, still far beneath the surface.
Fossat’s team deduced that the core of the Sun is rotating faster than the surface, taking one week to complete a rotation, compared with the surface’s 30 days. This is probably a remnant of the formation of the Sun itself as it accreted gas from its surroundings, absorbing its angular momentum.
And in 2020, researchers using the Borexino Neutrino Detector in Italy detected neutrinos from the CNO cycle within the Sun. This was the first confirmation that this energy source is indeed operating in the cores of stars and also occurs at all in lower-mass stars like our Sun.
The surface of the Sun
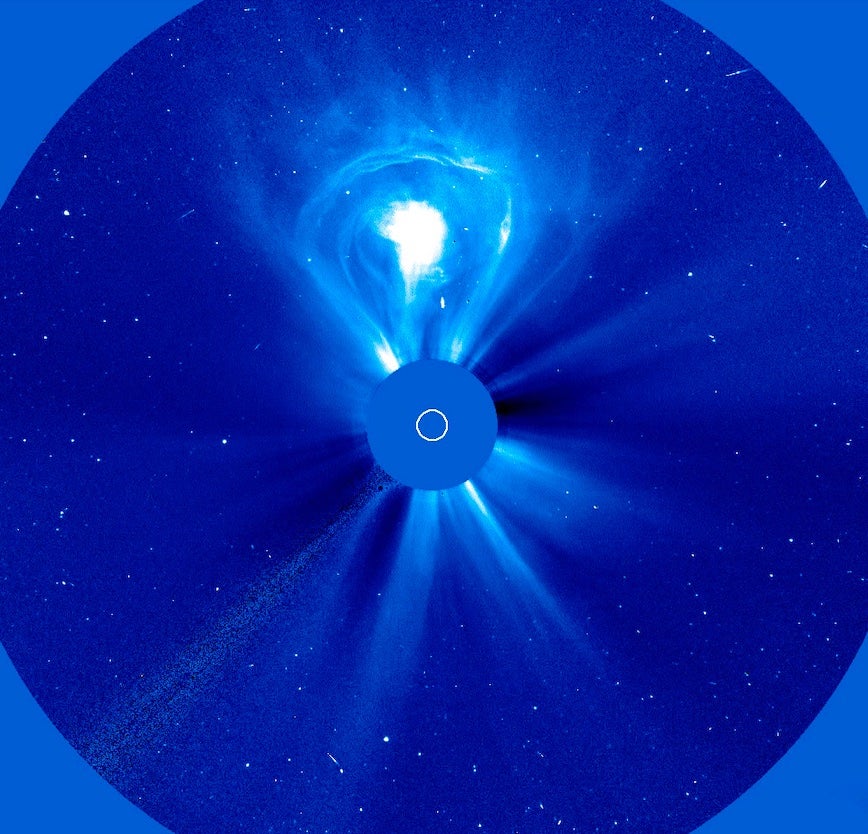
The photosphere, or visible surface of the Sun, is incredibly complex and dynamic, a seething surface of convection cells, tangled magnetic fields, and explosive flares and prominences. At virtually every scale, from feet to thousands of miles and from seconds to years, there are phenomena demanding explanation.
Among these are sunspots, observed since the first millennium b.c.e. By the 20th century, sunspots were identified as locations where the internal magnetic field of the Sun punches through the surface, causing the local plasma to shine at a lower temperature and appear dark against the brilliant surroundings. Their numbers rise and fall in a roughly 11-year cycle first discovered in 1843 by amateur astronomer Heinrich Schwabe. Since then, astronomers have tried to decipher this cyclical behavior. A clear explanation leading to reliable forecasts still eludes us, but pieces of the problem have been resolved.
Despite their surface-level appearance, sunspots originate in the Sun’s interior. Just below the photosphere is a convection zone, which reaches roughly one-third of the way to the center of the Sun. Within this region, plasma flows like a fluid, carrying heat and energy upward and sinking again once it has cooled. Beneath this sits the tachocline, a transition zone that separates the convective and radiative zones of the Sun. The deeper radiative zone reaches down to the core; within the radiative zone, the solar plasma rotates as if it were a solid body.
Within the tachocline, the differential rotation of the convection zone shears against the uniform rotation of the radiative zone, amplifying the embedded magnetic field. When portions of the magnetic field achieve buoyancy and rise to the surface, they form the sunspot groups we see.
Just as studying seismic waves on Earth allows geologists to probe its inner structure, SOHO’s observations of the oscillatory motions of the solar surface allow us to probe how the convection zone and tachocline have changed during the last three sunspot cycles. It seems that just as Earth has equatorial trade winds, the solar interior above the radiative zone has what is called meridional circulation. Plasma at the equator flows to the polar regions at about 45 mph (72 km/h), dives back to the tachocline, and then flows equatorward, completing one loop every 11 years. Changes in this flow speed seem to be correlated with the strength of the subsequent sunspot cycle — the faster the drift velocity, the stronger the sunspot cycle, as measured by the total area of the sunspots.
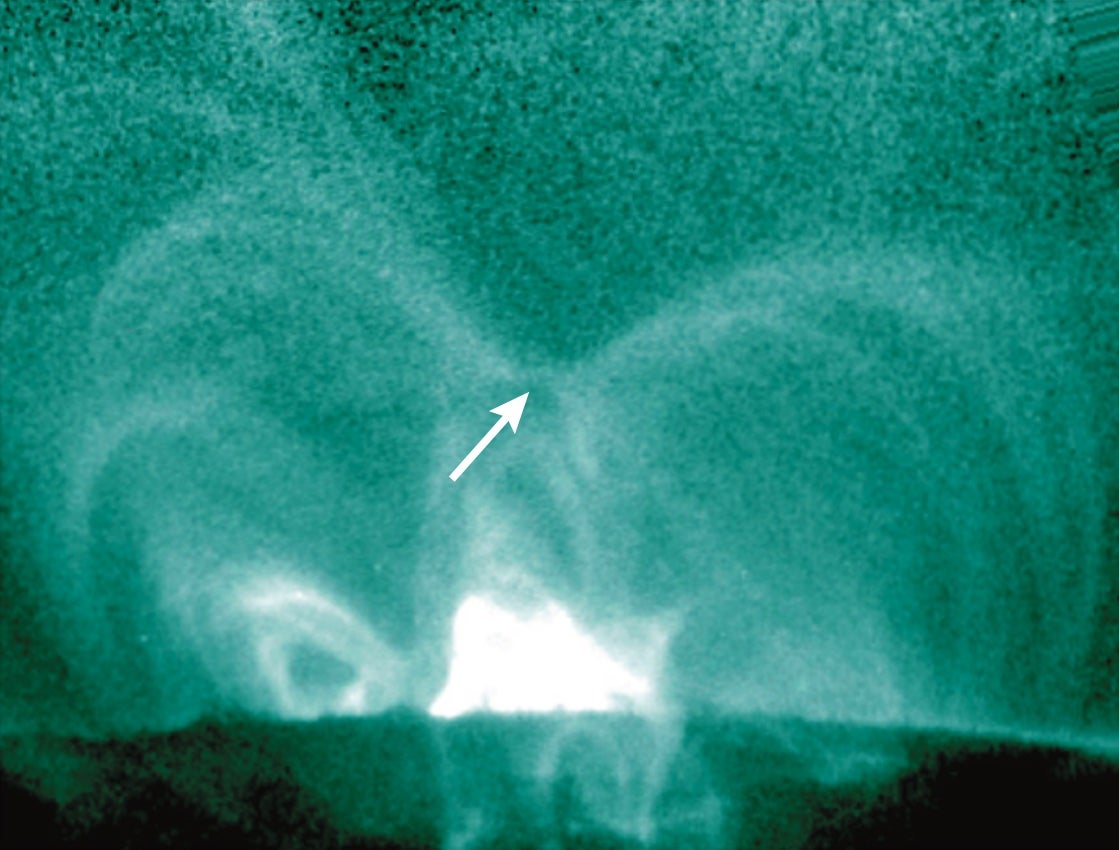
Seeking reconnection
On Sept. 1, 1859, English astronomer Richard Carrington was observing a group of sunspots when “two patches of intensely bright light broke out” from them, he later wrote. He had become the first observer to see a solar flare in real time. That night, the aurora borealis was seen as far south as Cuba and Colombia. Earth had been engulfed by the strongest geomagnetic storm ever recorded — one that, if it occurred today, could have catastrophic impacts on power grids and communications infrastructure.
Carrington’s serendipitous observation showed that the Sun is capable of unleashing tremendous amounts of magnetic energy from sunspots — and that energy can also have enormous impacts on Earth. But understanding the physics of how that actually happens has remained one of the Sun’s most elusive mysteries.
A breakthrough came in 1950, when British space scientist James Dungey first described in his Ph.D. thesis at the University of Cambridge a phenomenon called magnetic reconnection: When magnetic field lines in plasma are coiled and twisted, they store magnetic energy, like a wound-up rubber band. When the field lines break and then reconnect, they release this energy by converting the magnetic energy into other forms. This process goes a long way toward explaining how the solar plasma is heated and ejected in explosive flares. The theoretical details were explored by astronomer Peter Sweet and solar physicist Eugene Parker in 1956.
Magnetic reconnection became the canonical explanation for flares and other magnetic phenomena. However, it remained observationally unverified for decades, until space missions in 2008 and 2015 detected magnetic reconnection events in Earth’s magnetosphere and the subsequent aurorae they caused.
The Large Plasma Device at UCLA and the Magnetic Reconnection experiment at Princeton University can create magnetic reconnection events in the lab to study their detailed behavior. All of this research has abundantly confirmed the basic mechanism detailed by Sweet and Parker. Today, scientists use the concept of magnetic reconnection to investigate many systems in the universe, from bipolar outflows in star formation to the disks and jets of supermassive black holes.
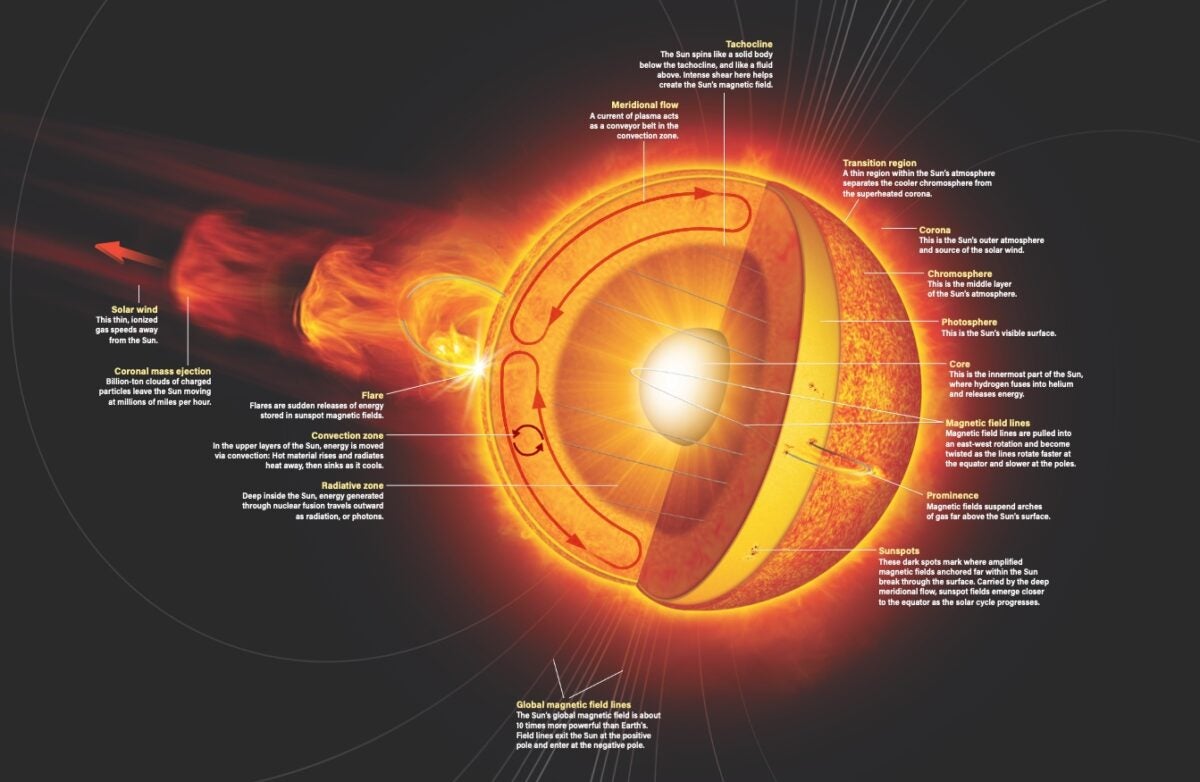
The corona
Magnetic reconnection has also emerged as a key to another of the Sun’s enduring mysteries: why the corona heats up even as it extends farther away from the Sun.
Our awareness of this conundrum dates to the mid-19th century, when spectra of the corona taken during total solar eclipses revealed emission at wavelengths never before seen, unassociated with any known element. It took the development of quantum mechanics in the 1920s to show that some of these lines were not emitted by new elements — they were produced by known elements that had been stripped of several of their electrons, a state only possible if the temperature of the corona was millions of degrees.
Yet the photosphere was an estimated 9,570 to 11,730 degrees Fahrenheit (5,300 to 6,500 degrees Celsius). So, a corona that was so much hotter seemed an impossible state that defied the laws of thermodynamics, much like an ice cube surviving in an oven. How can a hot, million-degree corona sit on top of a much cooler, thousand-degree photosphere?
In 1987, Parker proposed that the corona could reach such temperatures if it were heated by multiple, repeated small flares. This is just one of many theories for how events on the solar surface can heat coronal plasma, and perhaps all of them contribute. But in the last few decades satellite observations have detected explosive flares caused by magnetic reconnection in the inner corona and the transition region separating it from the chromosphere. These flares come in a variety of sizes, down to nanoflares first detected by JAXA’s Hinode in 2011, which can see features as small as 470 miles (760 km) across.
By combining data from two NASA satellites — the Interface Region Imaging Spectrograph (IRIS), which can image solar structures as small as 155 miles (250 km) every second, and the Solar Dynamics Observatory (SDO) — researchers have discovered that nanoflares undergo magnetic reconnection, leading to the rapid heating of the local solar plasma to millions of degrees. These events are so numerous they can easily explain why the corona reaches millions of degrees.
Coronal mass ejections (CMEs) are another feature of the corona, hurling billions of tons of plasma into interplanetary space. These wreak havoc with planetary magnetic fields upon impact and cause aurorae, magnetic storms, bursts of high-energy protons, and other effects that are harmful to astronauts and satellite technology. These explosions are also caused by magnetic reconnection in the corona (often accompanying flares in the photosphere). The entrained plasma is then hurled out into space at millions of miles per hour.
Extensive studies of CMEs by satellites both in situ and remotely observing from different angles (such as NASA’s STEREO A and B) have allowed us to understand how they are produced and propagate in space. When a CME is first ejected, a shock wave forms as it plows through the denser lower corona. This compresses the local magnetic field, creating a natural particle accelerator that amplifies the energies of plasma particles — mostly protons — by thousands of times. Traveling at nearly the speed of light, these protons arrive at Earth within a few hours and present a severe radiation hazard to astronauts and satellites. They can even be detected on the ground by instruments that normally monitor the flux of cosmic rays produced by distant black holes and supernovae.
Fortunately, CMEs can now be physically and mathematically modeled so that increasingly accurate forecasts can be made for what to expect near Earth.
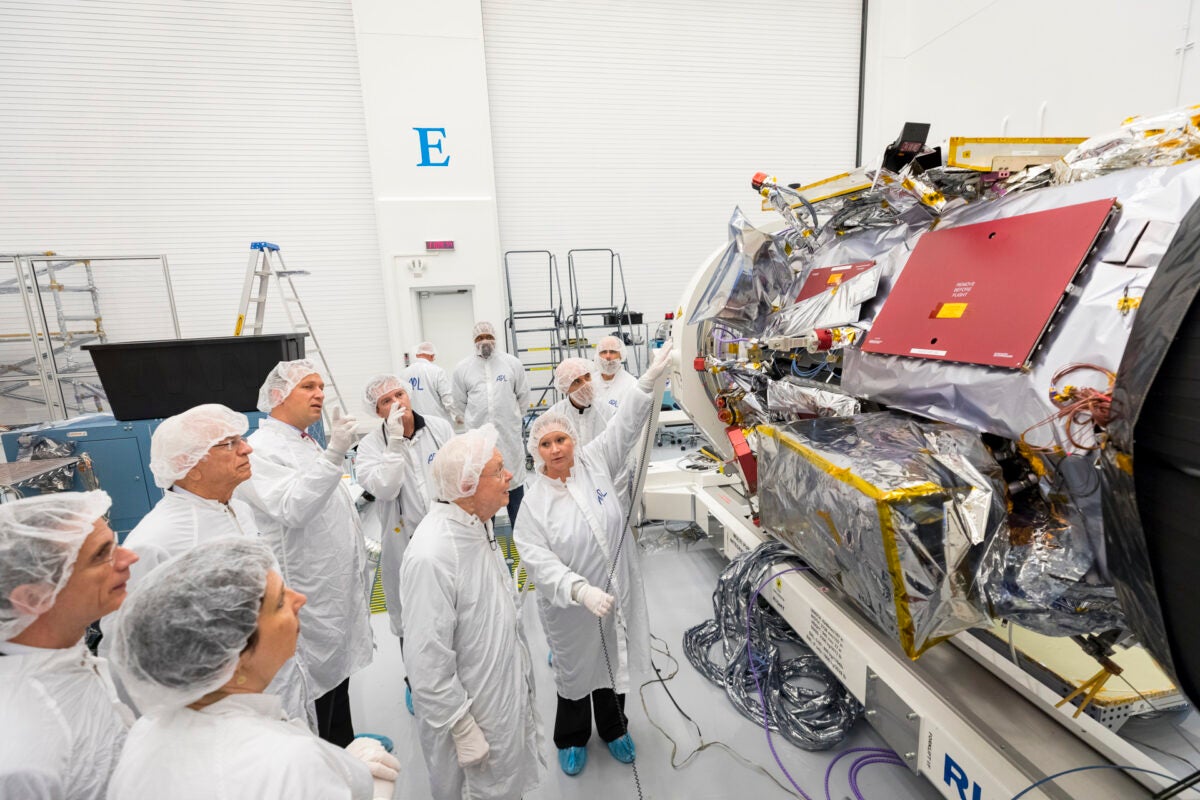
The solar wind
The corona may be the outermost visible layer of the Sun, but our star’s physical presence reaches far beyond that. In 1951, German astronomer Ludwig Biermann proposed that the shapes of comet tails were affected by a global stream of what he called “corpuscular radiation” from the Sun, and that the comet tails acted like windsocks. In 1958, Parker published the defining paper on what is now called the solar wind. Solar wind particles were subsequently detected by Mariner 2, launched in 1962.
There are two solar wind streams: a slow wind traveling at about 124 miles (200 km) per second and a fast stream at about 250 miles (400 km) per second. The slower wind is more persistent and correlates nicely with Parker’s model, while 1973 X-ray studies conducted on the Skylab space station found that the high-speed wind is associated with features called coronal holes. These holes occur where the magnetic lines of force from the corona open out into interplanetary space and act like pipes through which the hot coronal plasma can flow quickly outward.
But where does the solar wind originate? For decades, researchers had lacked direct observations of any proposed mechanism, including magnetic reconnection. But in recent years, this has changed dramatically.
Since 2018, NASA’s Parker Solar Probe has been detecting magnetic “switchbacks” in the outer regions of the corona, in which the local magnetic field rapidly changes direction. Scientists think this is a sign that magnetic reconnection is occurring closer to the Sun, as open and closed magnetic field lines interact and reconfigure themselves. These disturbances hurl globs of plasma into interplanetary space, though it’s unclear whether this material can account for the solar wind.
A separate line of evidence has emerged thanks to ESA’s Solar Orbiter, which launched in 2020 and approached the Sun to about 28 million miles (45 million km) in March 2022. At that distance, its Extreme Ultraviolet Imager was able to resolve the inner corona into a multitude of individual plumes and jets reaching upwards into the corona. These so-called picojets are only 62 miles (100 km) across and last just under 100 seconds, launching their plasma at 62 miles (100 km) per second. Future observations should provide a clearer picture of whether and how these jets might collectively generate the solar wind.
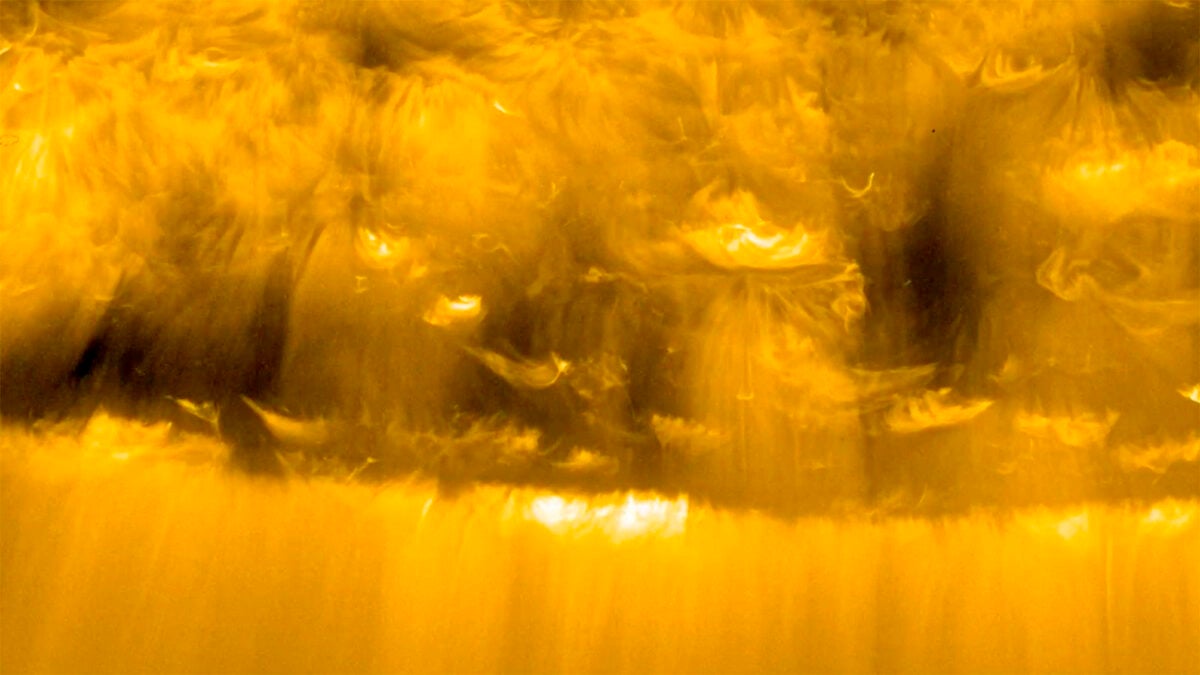
Many chapters left
So, where do we now stand?
As with Earth, the terra incognita of our star remains its interior. We only know what is generally occurring inside the Sun, while the details beg for study. Exactly what goes on in the vast radiative zone? Theory suggests a massive, primordial magnetic field there, dating to the Sun’s formation. How do sunspot cycles come and go and what determines how active they will be? Is it all just unpredictable chaos? How often does our Sun release massive superstorms like the 1859 Carrington Event, and what prompts them?
There are plenty more questions to ask, and our state-of-the-art space missions are uncovering new details with every orbit.