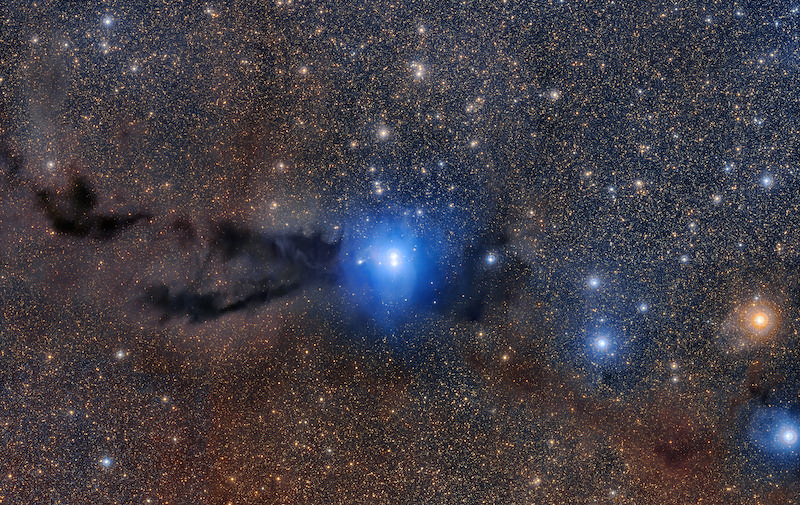
Adrift in a sea of dim red dwarfs, the Sun stands out like a bright yellow gemstone. Astronomers estimate these low-mass red dwarfs account for 70 to 80 percent of our galaxy’s stellar content. Why? Are we here to ask that question only because life arose on a planet circling a star several times the mass of its neighbors?
Stars are a fundamental building block of the cosmos. Their births, lives, and deaths profoundly affect the evolution of galaxies everywhere and the potential for life to develop on any planets born in the wake of a star’s formation. As such, they ultimately are the key to the universe’s long-term future.
“[But] the theory of stellar structure and evolution has a critical missing element: It is not able to predict the masses of stars that form in our universe,” says Charles Lada, a senior astrophysicist at the Harvard-Smithsonian Center for Astrophysics.
“The distribution of stellar masses at their birth, the so-called initial mass function (or IMF) is the most fundamental boundary condition that needs to be observationally determined to enable a complete and predictive theory of star formation to be constructed,” Lada writes in Astrophysics in the Next Decade: The James Webb Space Telescope and Concurrent Facilities. “With knowledge of the IMF and how this function varies in space and in time, one can, in principle, predict the future evolution of all stellar systems, from galactic star clusters to massive galaxies.”
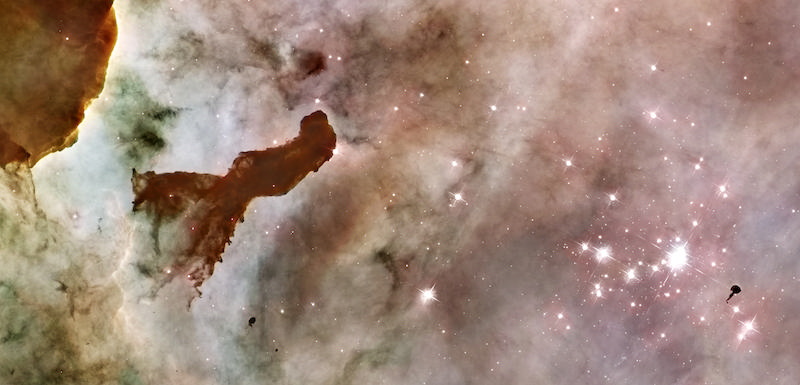
At the limit
Astronomers are arguably most puzzled by the fact that of the roughly 400 billion stars in the Milky Way, some 300 billion appear to be red dwarfs, also known as M dwarfs. Even more perplexing: By some estimates, the IMF peaks at around one-tenth of a solar mass. That’s awfully close to the minimum mass needed to ignite hydrogen fusion in the smallest red dwarfs, which is about 0.08 solar mass. Below that limit are brown dwarfs — failed stars without enough mass to fuse hydrogen into helium.
Stars form from the diffuse, cold interstellar gas that makes up molecular clouds. These clouds span roughly 100 light-years and hold between 10,000 and 1 million solar masses of material. “It is remarkable,” Lada writes in the same book, “that the physical process of star formation . . . through the action of gravity, transforms a small fraction of this material, reducing its size by more than eight orders of magnitude and increasing its density more than twenty orders of magnitude, into numerous objects with just the right mass to fuse hydrogen.”
Astronomers are still trying to figure out exactly how this happens. “Since we don’t yet have a complete theory of star formation, we don’t yet have an accepted theory of how the IMF was put together,” says Lada.
Understanding the IMF is important partly because stars of different masses play different roles in the life of a galaxy, says Chris McKee, an emeritus professor of physics and astronomy at the University of California, Berkeley. The more massive stars create heavy elements and energize the interstellar medium through their radiation, stellar winds, and supernova explosions. Their small siblings, however, are the ones that dominate the total mass in stars.
A star is born
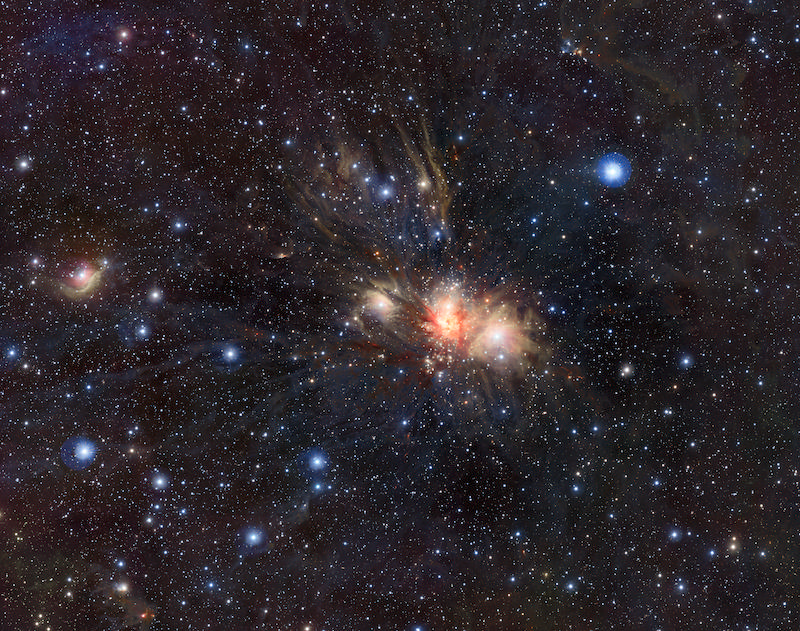
A collapsing molecular cloud will give birth to stars with a range of masses defined by the IMF. Research into the origin of the IMF began some 60 years ago. In 1955, Cornell University theoretical physicist Edwin Salpeter put forward the idea that stellar populations must have what he termed an initial mass function. Sometimes known as the Salpeter function, it describes the distribution of masses among a newly formed group of stars before any of them are able to lose mass or explode as supernovae.
Molecular clouds are cold — typically only about 10 kelvins — and their temperature is similar across a large range of densities, says astrophysicist Matthew Bate of the University of Exeter in the United Kingdom. The surprising thing about the IMF is that wherever we look in our galaxy, stars always seem to have the same distribution of stellar masses, he says.
And the typical stellar mass is just about the minimum mass at which nuclear fusion can occur, says Mark Krumholz, an astronomer at the Australian National University in Canberra. “The similarity between the peak of the IMF and the mass scale for nuclear fusion seems like it can’t be a coincidence, but it’s far from easy to come up with a physical explanation that links them together,” he says. After all, the interstellar medium from which stars form is a cold, low-density gas. Why should it know the first thing about nuclear fusion?
According to Lada, a molecular cloud’s collapse into stars can produce the IMF in two ways: turbulent fragmentation and thermal Jeans fragmentation. Supersonic turbulence moving through a giant molecular cloud drives turbulent fragmentation: The clouds break apart into filaments, dense sheets, and molecular cores, leaving behind low-density voids. In thermal Jeans fragmentation, a cloud fragments when the inward pull of gravity exceeds the outward force exerted by the gas’ thermal pressure. So cold, dense clouds are more apt to collapse than warmer, more rarefied ones. The latter process is named after the late British physicist Sir James Jeans, who showed that once a gas cloud reaches a critical mass, gravity will win out, and the entire cloud — or an individual pocket within the cloud — will fragment and collapse into dense cores.
Once a dense core with a mass equal to a few times the mass of the final star forms inside the molecular cloud, gravity triggers its collapse, and a protostar is born, says Patrick Hennebelle, an astrophysicist at France’s AIM/CEA Saclay. The protostar then accretes the rest of the parent core, he says. The initial masses of these dense molecular cores are governed by their own core mass function (CMF). And many researchers now think that understanding the CMF is the key to understanding the IMF’s origins.
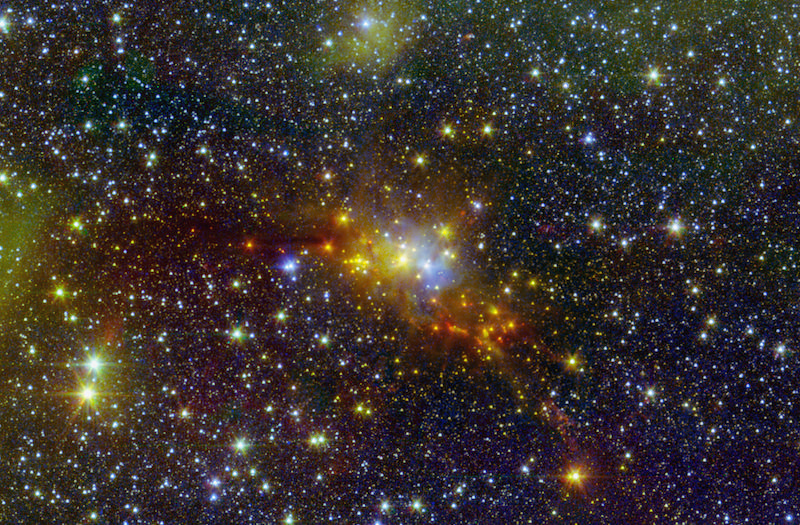
But the story grows more complicated when you consider that the core may fragment as it collapses, and the protostar may blow off excess material as it accretes. Does the CMF control the IMF’s shape? Observations show that at the very least, the two seem to have strong similarities.
“The dense core collapses until its central densities and temperatures become starlike and are high enough to begin nuclear burning,” says Lada. “That signals the birth of a new star.” He adds that it also begins the process of reversing the collapse of the remaining core material, enabling the star to emerge from its birth cloud.
We know that all low-mass stars form in dense cores that do not have much more mass than the stars they produce, says Steven Stahler, a theoretical astrophysicist at UC Berkeley. So the real question, he says, is why does nature create dense cores with stellar-type masses?
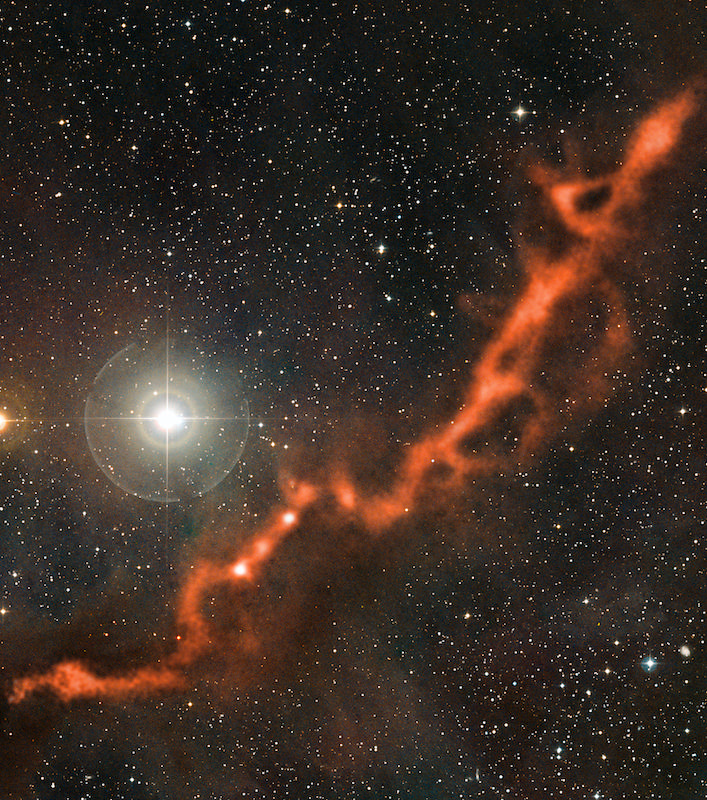
Pulling the trigger
The bulk of star formation in the Milky Way occurs in a relatively thin disk and within 30,000 light-years of Sagittarius A*, the supermassive black hole at the galaxy’s center. Astronomers estimate that 15,000 stellar nurseries populate our galaxy — and they all started as cold molecular clouds. In most cases, large-scale density waves moving through the spiral arms triggered the collapse of these clouds. But the shock wave from a supernova or a stellar bubble expanding from a high-mass O- or B-type star also can trigger fragmentation into stellar cores.
To date, astronomers have detected only a small variation in the mass distribution of stars. “This strongly argues for some process of regulation that decides what mass stars should have,” says Krumholz.
What might that process be? Higher-mass molecular cores are too massive to balance gravity and thermal pressure, and large turbulent motions and higher internal pressures usually characterize them, says Lada. “When such turbulent objects collapse, they can fragment and form multiple protostellar cores which then evolve to become binary [stars].”
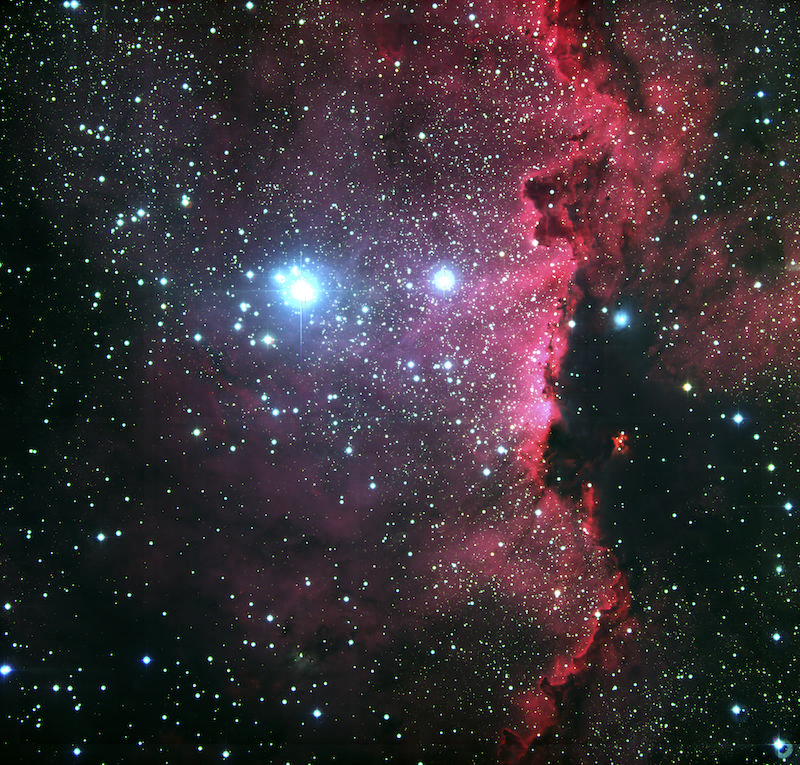
He adds that the more massive and turbulent the core, the more readily it can fragment. That might explain why massive stars are more likely to be found in binary systems than low-mass stars.
But Bate thinks that feedback from the radiation emitted by low-mass protostars is one key to determining the IMF. He contends that the radiation heats the gas in the surrounding molecular cloud, which inhibits further fragmentation.
A universal IMF?
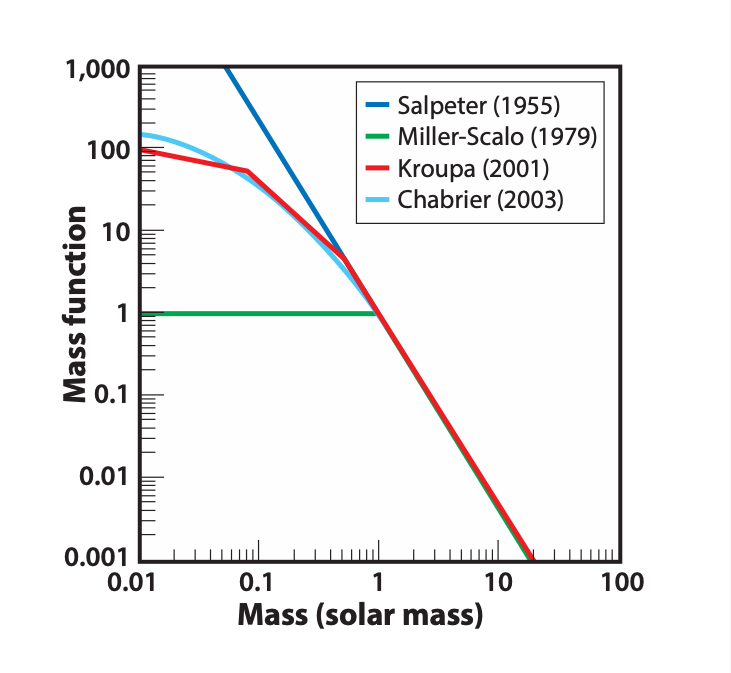
Of course, the best observations of the IMF come from relatively nearby star-forming regions in the Milky Way. But as far as we know, the IMF in disk galaxies like ours is universal, says McKee. One of the big questions confronting astronomers is whether the IMF remains constant over both space and time.
“So far, there is no evidence to suggest that the IMF has significantly varied over cosmic time,” says Lada. Still, there might be an exception in the earliest stages of the universe. Astronomers believe that the first stars, so-called Population III stars, were the most massive and possessed the fewest metals. (Astronomers refer to all elements heavier than helium as “metals.”) Once these early stars started churning out heavier elements in earnest, however, subsequent generations of stars incorporated these metals, and the typical stellar mass dropped significantly.
Bate says it is still unclear whether the IMF ever varies. He wonders whether the mass function would be the same even in the extreme environments found near the centers of massive galaxies, where radiation must be much higher, or early in the universe, when there was no interstellar dust.
A few observations hint at the possibility of different IMFs. In a 2009 paper published in the Monthly Notices of the Royal Astronomical Society, Bate writes that observations of stars orbiting Sagittarius A* show the IMF near the galaxy’s center may be “top heavy,” biased toward massive stars. The same goes for stars in the Arches Cluster, the Milky Way’s densest-known open cluster, which lies some 25,000 light-years from Earth in the constellation Sagittarius. Even so, he notes that the apparently top-heavy IMF in the Arches Cluster may be due to its evolution rather than to the conditions at its birth. The opposite effect may be happening in the crowded cores of giant elliptical galaxies, where indirect observations point to an excess of low-mass stars.
The differences in the amount of metals throughout the universe and over time seem to have a relatively small effect on the IMF. “You can go to the Small Magellanic Cloud where there is about a fifth of the dust and metals found in the Milky Way, and [you find] no detectable difference,” says Krumholz. According to McKee, you wouldn’t expect to see much effect as long as the metal content is greater than about a few ten-thousandths of the current value. He says the main difference between the metal-free first stars and those being born today is that the earliest ones were born in a framework dominated by dark matter, while current ones form due to their own self-gravity.
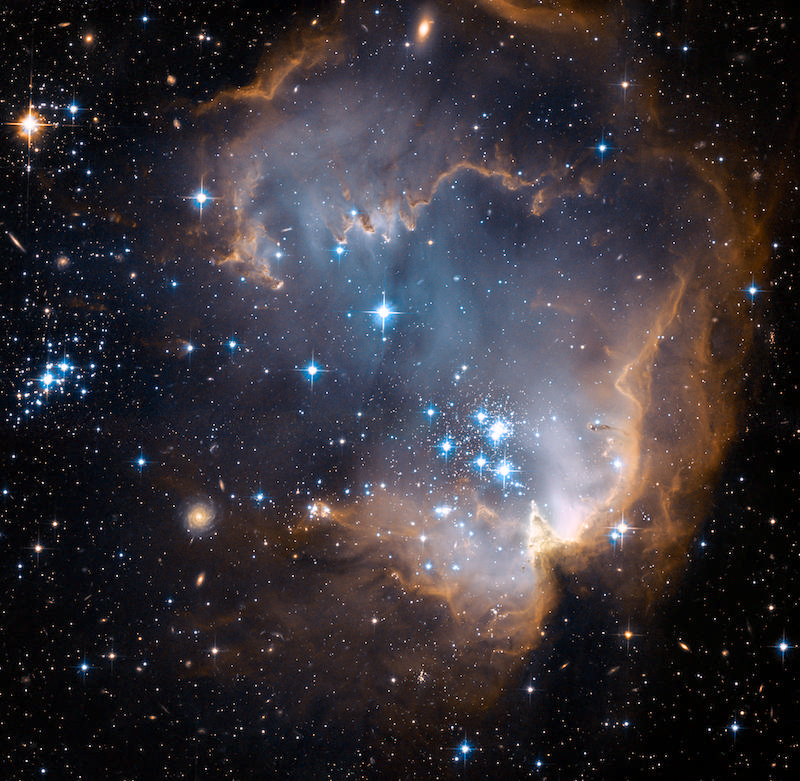
New tech, new answers
New and improved computer modeling will help theorists refine their calculations of the IMF, researchers say. But observational improvements could prove even more important. The European Space Agency’s Gaia satellite is allowing astronomers to hone their mass estimates for stars in distant clusters. And NASA’s James Webb Space Telescope will allow astronomers to directly observe the IMF in such clusters. It also will reveal the IMF in some galaxies with more extreme conditions than our own.
On the ground, the Large Synoptic Survey Telescope and the European Extremely Large Telescope, both now under construction in Chile, should provide the light-gathering ability and resolution to advance our knowledge of the IMF and any possible variations in it.
And the Atacama Large Millimeter/submillimeter Array (ALMA) will make observations of dense interstellar regions heretofore obscured by dust. A primary assumption in current theory is that the distribution of masses of gravitationally bound regions within molecular clouds determines the IMF, says McKee. “ALMA is the ideal instrument to test this, particularly for high-mass stars,” he says.
However, Lada points out that we don’t yet have the technology to build some of the telescopes needed to produce high-angular-resolution maps of the gas and dust across entire molecular clouds. Ideally, this would require millimeter- and submillimeter-wave telescopes with apertures of 50 to 100 meters, and spectroscopic cameras capable of multimillion-pixel resolution.
“We know how to build the [telescopes] but are far from realizing the technology to produce millimeter and submillimeter cameras with spectroscopic capability and millions — or even hundreds — of pixels,” says Lada. With such capability, we might learn how molecular clouds form and evolve to produce the core mass function that in turn produces the IMF, he says.
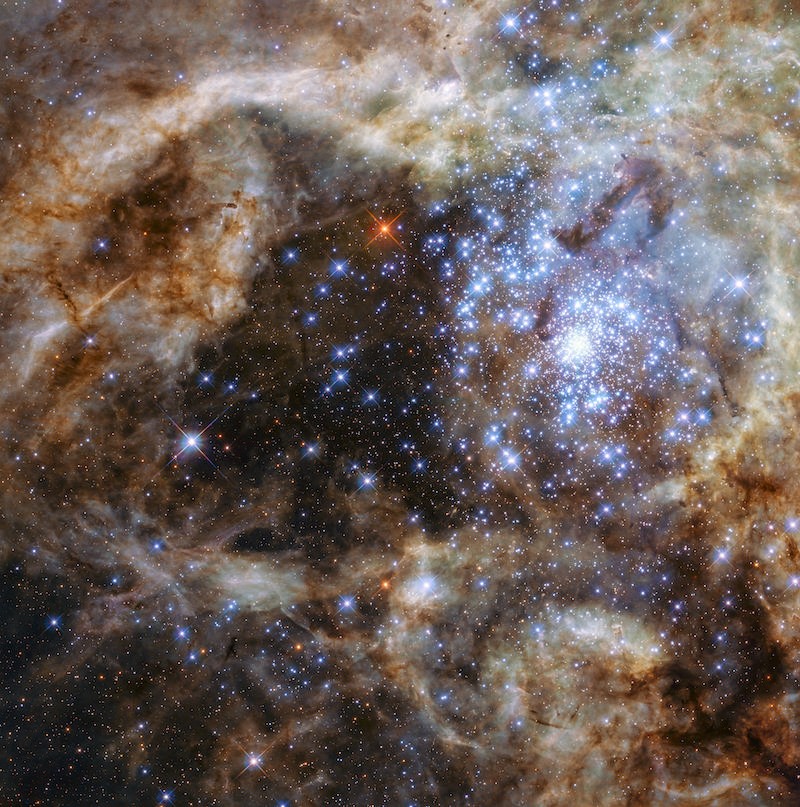
An intriguing mystery
Ultimately, when it comes to understanding why nature produces such an abundance of low-mass stars, astronomers are left with more questions than answers. Why doesn’t the IMF lend itself to massive O-type stars, brown dwarfs, or even gas giant planets?
Of course, as inhabitants of a solar system dominated by what appears to be a rather ordinary G-type yellow dwarf star, the observed IMF may be precisely why we are here to ask such questions. If the IMF were honed to produce only brown dwarfs or gas giants — or short-lived, massive O-, B-, or A-type stars — then the road toward life would have been much tougher.

Still, yellow dwarfs are not the IMF’s preferred outcome, either. The small M dwarfs that the IMF favors have life spans of 50 billion to 100 billion years, enough time for life to arise and re-arise numerous times on any Earth-like planet that might circle such a star. In contrast, our Sun already has made it halfway through its life as a stable star, and its endgame as a dying red giant promises to be quite messy.
Tiny red dwarfs are probably not the best stars to host planets on which life might evolve, however. After all, they tend to be much more active than the Sun, particularly early in their lives. Potentially life-hindering coronal mass ejections and strong stellar flares likely would occur within striking distance of any extrasolar planet that might be habitable. So, it’s probably a stretch to say the IMF is fine-tuned for life as we know it.
Yet unraveling the IMF’s complexities remains crucial to researchers in almost every field of astronomy and astrophysics. With the advent of new telescopes and technologies in the coming years, perhaps the IMF won’t be such a mystery to astronomers of future generations.